Neutrino Oscillations
Neutrino oscillations were first proposed by Pontecorvo in 1967 who suggested that neutrinos change their flavour as they travel from the Sun to the Earth. On the one hand, it seemed to be a great explanation of the significant deficit of solar neutrinos, since all the existing experiments at that time were predominately sensitive to only electron neutrinos. But in 1967, there was no known mechanism included in the standard model that would allow neutrinos to oscillate between different flavours.
Today, the proposed mechanism of neutrino oscillation extends the Standard Model by assuming that neutrinos do have mass. It is hypothesised that neutrinos have two fundamental sets of states, one set that accounts for the neutrino flavour and has well-defined weak interactions (weak eigenstates, denoted νe, νμ, ντ) and one set that has well-defined masses (mass eigenstates, denoted ν1, ν2, ν3). These two sets get mixed, which means that, for example, a particle which is created as an electron neutrino has a certain probability of being observed subsequently as a muon or tau neutrino. This mixing means that each neutrino mass state is a mixture of the different flavour (weak) states, and vice versa.
According to quantum mechanics, all particles have an associated wave nature. The waves describing the travelling neutrinos oscillate in and out of phase. The masses of the different neutrino mass states are not the same, and so within a given flavour state, the different mass states travel at different speeds. But remember that within each mass state there are different flavour states. Because the different mass states are travelling at different speeds, the flavour states in one mass state have a different wavelength compared with flavour states in another mass state; hence, the wavelength of their associated probability wave is different. An originally pure muon neutrino beam can oscillate into an electron neutrino beam and back over space and time. This identity change and the distance over which it takes place depends firstly on the neutrino energy and secondly on the two wavelengths, which themselves depend on the difference between the squares of the masses of the neutrinos.
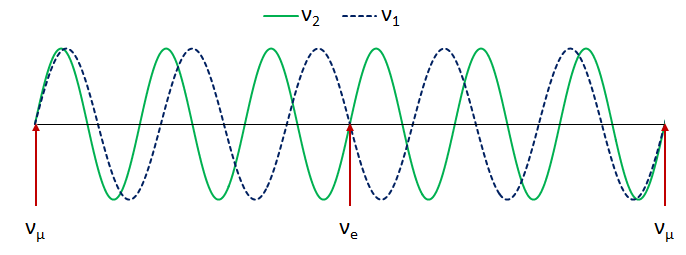
T2K
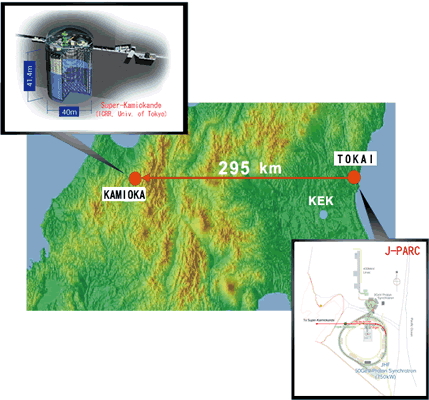
Two of the current goals of neutrino physics are to understand the origin of neutrino masses and to measure the parameters that describe neutrino mixing. The next big neutrino experiment is the T2K experiment in Japan. It developed into a major international collaboration with collaborators from 12 countries. The main purpose of the experiment is the measurement of the unknown mixing angle from the observation of the νμ to νe oscillation. Two main neutrino oscillation measurements will be made at T2K: the disappearance of muon neutrinos from the original beam and the appearance of electron neutrinos. T2K consists of three main components: a proton accelerator at J-PARC which produces the neutrino beam; a suite of near detectors at J-PARC which measure the properties of the neutrinos before they've had a chance to change flavour; and a far detector, Super-Kamiokande, which measures the neutrino properties 295 km later. The beam itself that will be used is the most intense artificial neutrino beam that has so far been constructed and it will be produced at the J-PARC facility on Japan's east coast. The beam will be directed underground to the Super-Kamiokande detector some 295 km away.
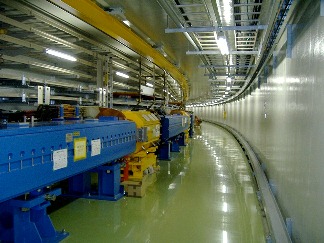
The beamline at T2K
The beam is created by colliding a proton beam with a suitable target
and collecting and focusing the pions into a decay volume. Muon neutrinos
and muons are the subsequent decay products. The decay volume length is
chosen so that most of the pions but very few muons will decay before reaching
the beam dump at the end. The beam dump stops all particles except the muons
and muon neutrinos. The beam then carries on through 150 m of rock, which
stops the muons, leaving a pure neutrino beam. The muon neutrino disappearance
measurement from the original beam is made by comparing the flux and energy
spectra at a near detector before oscillations, with that at the far detector,
Super-Kamiokande, after oscillations. The beam neutrinos detected in the
T2K experiment can be distinguished from background neutrinos due to the
Sun or other background sources by their energy spectrum, timing and direction
of travel. Comparison of the numbers of electron and muon neutrinos detected
in T2K allows the mixing angle to be measured.
Feynman diagrams
Details of the neutrino interactions with the nucleus creating muons and electrons. In the diagrams, the initial state is on the left and the final state is on the right. In the charged-current weak interactions shown, each initial-state neutrino produces a final-state charged lepton of the same generation; for example, an initial-state muon-neutrino will produce a final-state muon whereas an initial-state electron-neutrino will produce a final-state electron.
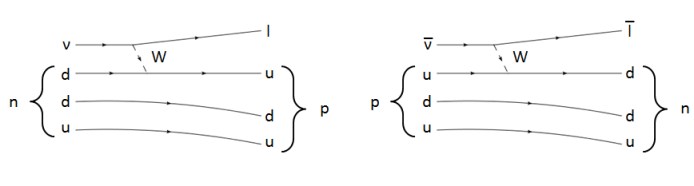
Feynman diagrams for neutrino interactions